The Claus Process
Refineries, gas processing plants and various chemical manufacturing facilities may produce H2S and other sulfur-containing compounds in such high quantities that they must be removed. Amine units or other processes are often used to remove the H2S from various streams and dispose of it, along with CO2, water vapor and other compounds, into a byproduct stream known as acid gas.
Other sulfur-bearing byproduct gases, such as sour water stripper gas containing ammonia, may also require removal from the facility. The acid gas and sour water stripper gas are fed to a facility that converts the sulfur compounds into a valuable product that can be safely handled. There are numerous processes for converting the sulfur compounds in these byproducts to elemental sulfur. Of these, the Claus process produces the greatest quantity of elemental sulfur.
The Claus process generally consists of the thermal, or combustion, section and the catalytic section.
1) Thermal or Combustion Section
This section typically involves a burner, a refractory-lined combustion chamber, a waste heat boiler and a sulfur condenser. This is where most of the reaction’s heat is released.
- Approximately ⅓ of H2S is burned to create SO2 and water:
H2S + 3/2 O2 → SO2 + H2O - Approximately 6% of the H2S thermally cracks to create sulfur and hydrogen:
H2S ↔ 1/2 S2 + H2 - Up to 60% of the remaining H2S reacts with SO2 to create elemental sulfur:
2 H2S + SO2 ↔ 3/2 S2 + H2O
Hydrocarbons and other combustibles in the feed are burned. The resulting hot gas is cooled by steam from a boiler. Cooling condenses the elemental sulfur.
2) Catalytic Section
This section usually has three catalytic stages, each consisting of a feed heater, a catalyst bed and a sulfur condenser.
- Gas from the thermal section is reheated and routed through a catalyst bed.
- SO2 from the thermal section reacts with unburned H2S to form elemental sulfur (generally known as the “Claus” reaction):
2 H2S + SO2 ↔ 3/X SX + H2O - Cooling from generating low-pressure steam condenses the sulfur.
- After the sulfur condenses, the gas can be reheated and routed through another catalyst bed.
Two Common Sulfur Plant Configurations: Straight-Through and Split-Flow
Abbreviations used in the diagrams below are as follows: AG = Acid Gas; SWSG = Sour Water Stripper Gas; TR = Thermal Reactor; WHB = Waste Heat Boiler; CDSR = Sulfur Condenser; RH = Reheater for a Catalyst Bed; CatBed = Catalyst Bed (Reactor).
A straight-through plant typically uses feed gas H2S concentrations above 50 mol% (wet). All acid gas feed goes to the burner and thermal reactor in a straight-through plant. When the burner exits directly into a large-diameter boiler flue, a minimum adiabatic flame temperature of 1700°F must be maintained.
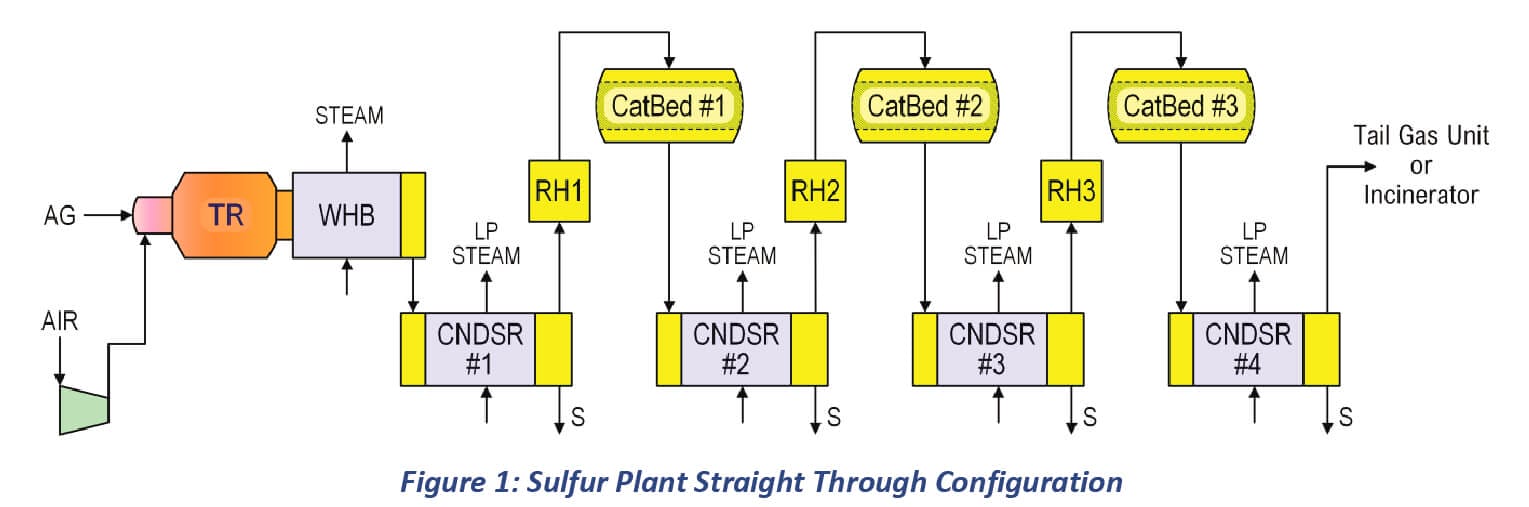
A split-flow plant is generally used when the feed gas is between 20 and 50 mol% H2S (wet basis). In a split-flow plant, 50-65% of the acid gas bypasses the burner and thermal reactor. All the combustion air needed for the process is still fed directly to the thermal reactor burner. This split-flow configuration allows the thermal reactor to operate at a higher temperature because a more significant fraction of its feed H2S burns to create SO2.

The acid gas fed to the thermal reactor must be large enough to ensure no unconsumed oxygen escapes the flame. Thus, some unburned H2S will exit the thermal reactor. The bypassed acid gas is added to the thermal reactor effluent after the waste heat boiler. The H2S/SO2 ratio entering the first catalyst bed is close to 2.0. Figure 2 above does not indicate a sulfur condenser immediately after the waste heat boiler. However, some split-flow plants include a sulfur condenser there. Split-flow plants with both acid gas and combustion air preheat can reportedly operate with H2S concentrations as low as 10 mol% H2S (wet).
Thermal reactors should be operated to maintain an actual temperature of at least 1450°F. Operating straight-through plants on acid gas containing H2S as low as 30 mol% (wet) may be feasible if both the acid gas and combustion air are significantly preheated.
Figures 1 and 2 show all catalyst beds in separate reactor vessels and all sulfur condensers as separate items. For plants smaller than approximately 125 LTPD, all three catalyst beds are often in a common horizontal reactor vessel. In plants up to approximately 300 LTPD, sulfur condensers can all be found in a common exchanger, with all tubes in the same shell.
The thermal reactor waste heat boiler often creates 400 psig or higher pressure steam to heat the catalyst bed feeds or drive air blower turbines. However, the waste heat boiler can generate the same low-pressure steam as the sulfur condensers (~50 psig) if other means are used to heat the reactor feeds. Some smaller plants have six tube passes in the same shell: three after the thermal reactor, and one after each of the three catalyst beds.
Claus Chemistry
The reactions described on Page 1 are a simplification of multiple reactions taking place in a Claus unit. Side reactions involve hydrocarbons, H2, CO and CO2 in the feed and intermediate gases. Other sulfur compounds (mostly COS and CS2) are also formed. Elemental sulfur occurs in multiple species, depending on temperature and pressure (S2, S3, S4, S5, S6, S7, and S8).
Burner and Thermal Reactor
(sometimes called a Reaction Furnace or a Muffle Furnace)
H2S and other compounds in the feed gases (acid gas or sour water gas) are partially burned. Some reactions are as follows:
H2S burning to SO2 (highly exothermic): H2S+3/2 O2 →SO2 +H2O
Claus reaction – endothermic at higher temperatures, when producing mainly S2: 2 H2S + SO2 ↔ 3/2 S2 + H2O
H2S dissociation, which is endothermic. Hydrocarbon burning reactions (high hydrocarbon content, especially C4+, olefins and aromatics, can result in soot or coke formation): H2S ↔ 1/2 S2 +H2
Hydrocarbons burning to CO: CnH2n+2 + 2n+1/2 O2 → n CO + (n+1) H2O
Hydrocarbons burning to CO2. Mostly occurs with O2 remaining after H2S is burned, since H2S oxidation is a faster reaction: CnH2n+2 + 3n+1/2 O2 → nCO2 + (n+1) H2O
Water gas shift reaction: CO+H2O ↔ CO2 +H2
COS formation: CO2 +H2S ↔ COS+H2O
CS2 formation: CO2 + 2 H2S ↔ CS2 + 2 H2O
NH3, if present. Destruction with SO2 is dominant. Some NH3 can react with O2, if free O2 is present. (See the Ammonia Destruction section on page 8): 2 NH3 + SO2 → N2 + H2S + 2 H2O
HCN, if present. Destruction with SO2 is dominant: 2HCN+SO2 →N2 +2CO+H2S
Some HCN reaction with O2, if free O2 is present: 2 HCN + 3/2 O2 → N2 + 2 CO + H2O
It is essential that any entrained water is stopped from entering the burner. Adequately sized feed K.O. drums must be included for the acid gas and sour water stripper gas feeds. Piping must not be pocketed downstream of the K.O. drums and is often insulated to prevent condensation. Sour water stripper gas lines are also well traced to keep them hot and to avoid condensation and ammonia salt formation.
Heat Exchange Equipment
Waste Heat Boiler
S2 ↔ 1/3 S6 ↔ 1/4 S8
Sulfur redistribution – exothermic when forming larger molecules while the temperature cools. S3, S4, S5 and S7 will also be present.
2 H2 + S2 ↔ 2 H2S
Some recombination of H2 and sulfur occurs near the hotter end of the boiler tubes. Generally, the net dissociation of H2S in the combined thermal reactor plus waste heat boiler is 5% to 8%.
Reheaters
1/4 S8 ↔ 1/3 S6 ↔ S2
Heating causes some redistribution of sulfur to smaller molecules.
Condensers
1/6 S6 ↔ 1/8 S8 ↔ S Liquid
S2, S3, S4, S5 and S7 will also combine. Endothermic when forming larger molecules.
Catalyst Beds
After cooling and sulfur condensing, the remaining gas is reheated and sent through a catalyst bed. The catalyst is usually activated alumina, sometimes titania. Before the mid-1970s, activated bauxite was commonly used.
The Claus reaction is exothermic at the lower temperatures here, where x averages about 6: 2 H2S + SO2 ↔ 3/X SX + H2O
Redistribution – (others include S3, S4, S5 and S7): S2 ↔ 1/3 S6 ↔ 1/4 S8
COS hydrolysis – mostly in a hot, active first bed: COS + H2O ↔ CO2 + H2S
CS2 hydrolysis – mostly in a hot, active first bed: CS2 + 2 H2O ↔ CO2 + 2 H2S
When n > 3, soot formation is possible. Olefins & Benzene are likely to soot: CnH2n + 2 → n C + (n+1) H2
Some of this “water gas shift reaction” can occur in a hot, active first catalyst bed. This results in a small decrease in CO due to no more than about 20% of equilibrium conversion. This also results in a small increase in temperature rise through the bed. This reaction can usually be safely ignored for catalyst bed simulations: CO + H2O ↔ CO2 + H2
Guidelines for catalyst bed feed temperatures are as follows:
First Bed: This bed is hot to promote the hydrolysis of COS and CS2 to H2S (see above reactions). Running with a feed temperature that results in a bottom-of-bed temperature of 590°F to 620°F is recommended. A first try for design feed temperature is 430°F to 440°F. It is recommended that the vessel walls containing the first bed be refractory-lined. This minimizes high-temperature sulfidation of the steel vessel walls during regular operation and protects those walls in case of catalyst bed sulfur combustion during startup and shutdown operations. Such sulfur combustion most often occurs in the first bed.
Second Bed: Generally, this bed is heated enough to ensure the reacting gas stays at least 30°F (17°C) above the sulfur dew point. This provides a safe margin to prevent sulfur from condensing in the catalyst pores. For the second bed, it is rare for the closest approach to the dew point to be at the top or bottom of the bed. Therefore, the dew point approach to actual temperature should be calculated for 0 to 100% reaction completion at 10% to 20% intervals through the bed. For a typical refinery sulfur recovery unit (SRU), the closest approach occurs at 50% to 80% of reaction progress. A good first try for design feed temperature is about 415°F.
Third Bed: As with the second bed, it is important to stay at least 30°F (17°C) above the sulfur dew point. For the third bed, the closest dew point approach usually occurs at the bed outlet. However, it is still a good idea to check the approach to dew point at 10% to 20% reaction completion intervals. Such dew point considerations can result in a recommended third-bed feed temperature as low as 375°F. However, a somewhat higher temperature can result in a slight recovery improvement for an aged third-bed catalyst. Commonly, a minimum third bed feed of 390°F to 400°F is used.
Bed Feed Temperature Requirements for Shutdown
When preparing to shut a sulfur plant down, catalyst beds are heated up to drive off elemental sulfur that is inside the catalyst pores. Depending on the shutdown procedure, the catalyst bed feeds will be heated up to at least 420°F prior to decreasing the acid gas feed. If the reactor feed is too cold, it will not drive off enough sulfur, and combustion of that sulfur will likely start during later stages of the shutdown when natural gas is burned with an excess of air in the burner. This is most often a problem with the third bed when its reheater has only been sized for normal operation. A third reheater smaller than the second reheater could be a problem, because that smaller third reheater may be unable to heat the third catalyst bed sufficiently prior to a shutdown. If electric reheaters are only capable of duties needed for normal operation, that could also result in insufficient heating prior to a shutdown.
Incinerator
As a minimum, tail gas exiting a Claus sulfur plant should be incinerated to oxidize the remaining H2S and other sulfur compounds to SO2. Such incinerators are supplementally fired with fuel gas to achieve an incineration temperature of about 1200°F. If there is also a need to oxidize CO to CO2, it is operated to incinerate at a minimum of 1500°F.
Reactions in the incinerator are as follows:
Burning H2S is the main purpose of an incinerator: H2S+3/2 O2 →SO2 +H2O
Burning elemental sulfur: Sn +n O2 →n SO2
Burning COS: COS+3/2 O2 →CO2 +SO2
Burning CS2: CS2 +3O2 →CO2 +2SO2
Up to 3% of SO2 further oxidizes to SO3: SO2 +1/2 O2 →SO3
Dilute sulfuric acid can condense on steel surfaces below approx. 350°F – very corrosive: SO3 + H2O ↔ H2SO4 (aq)
Fuel gas burning in the incinerator burner: CnH2n+2 + 3n+1/2 O2 → n CO2 + (n+1) H2O
Bad burners or insufficient air can make some CO: CnH2n+2 + 2n+1/2 O2 → n CO + (n+1) H2O
Hydrogen burning – mostly at T > 1250 °F: H2 +1/2 O2 →H2O
Little CO burns below about 1350°F. Incinerators operated to destroy CO generally run at a minimum of 1500°F: CO+1/2 O2 →CO2
Sufficient air should be fed to the incinerator to result in 1.5% to 2% O2 in the gas exiting the incinerator. Some operators target for 3% or higher O2 to allow for upsets.
Most often, the Claus plant tail gas is sent through a clean-up unit where more sulfur is removed before being sent to an incinerator or vented.
Methods for Heating Catalyst Bed Feeds
In both the straight-through and split-flow configurations discussed above, the process gases are cooled in sulfur condensers. After a sulfur condenser, the remaining gas must be reheated before entering the next catalyst bed. Methods of accomplishing this reheat are discussed below.
1. Direct Reheaters
Direct reheaters were often used in the past because of their relatively low cost. These are more common in very large sulfur plants. Following is a discussion of acid gas-fired in-line burners and fuel gas-fired in-line burners.
1.1. Acid Gas-Fired In-Line Burner
This requires a small slipstream of acid gas to be bypassed around the main thermal reactor burner. This results in a small decrease in recovery: ~0.1% for first bed reheat, ~0.2% for second bed reheat, and ~0.6% for third bed reheat. These burners are fired with 50 to 70% of stoichiometric air to minimize the chance of O2 breakthrough.
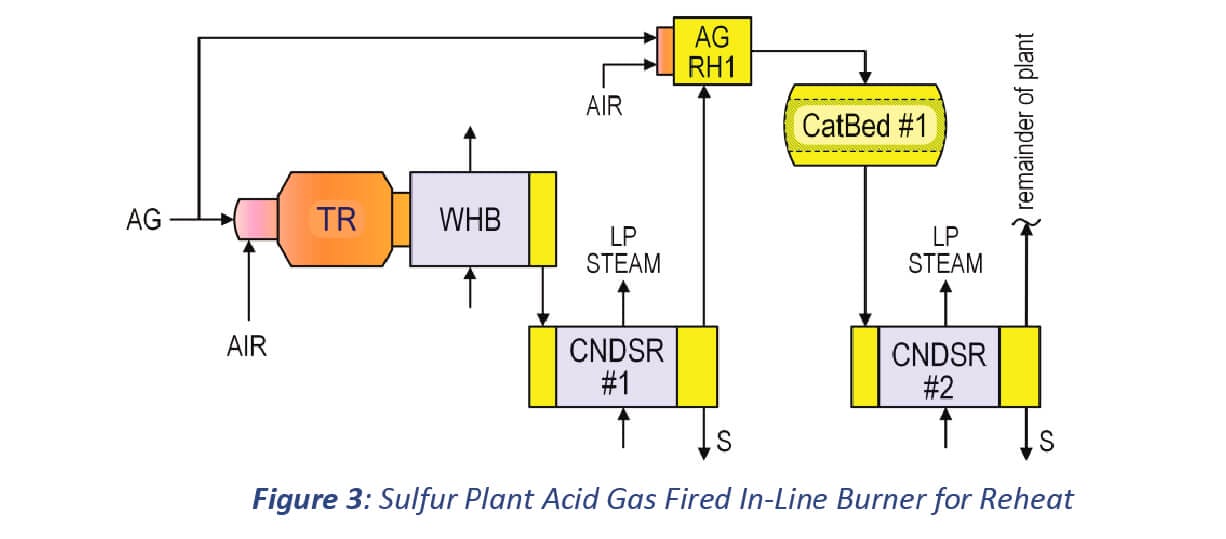
1.2. Fuel Gas-Fired In-Line Burner
When the acid gas is lean on H2S, fuel gas may be used as the in-line burner fuel. If done properly, the use of fuel gas for reheating the second or third catalyst bed feed can give slightly better recovery than reheating by burning acid gas.
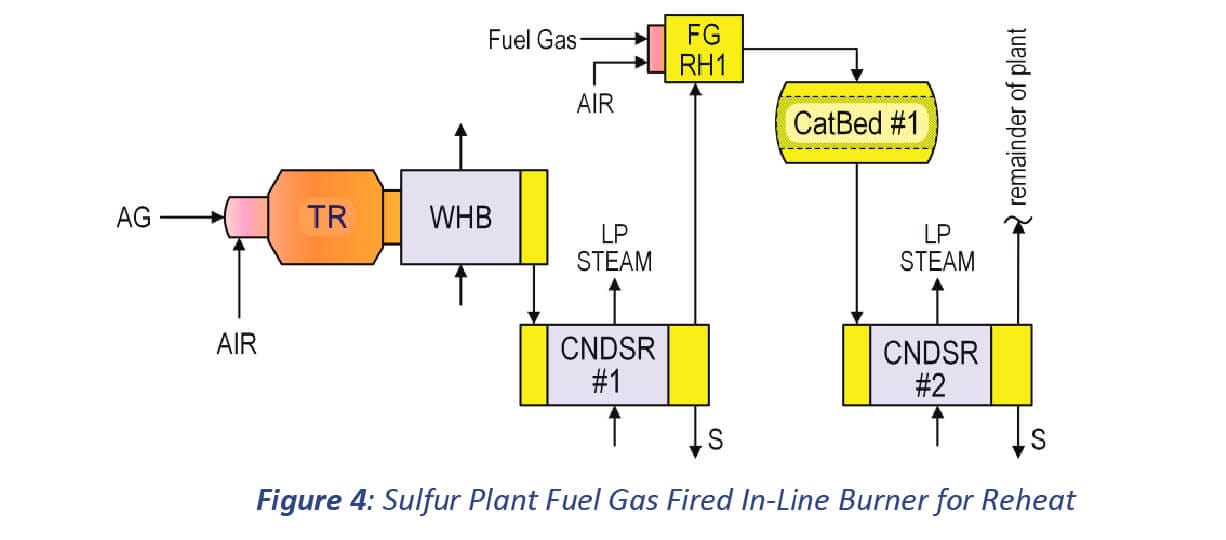
The fuel should be natural gas burned with 80-90% stoichiometric air. Traces of oxygen escaping the flame will slowly deactivate the catalyst, especially at lower catalyst bed temperatures. Thus, the use of fuel gas for firing a reheater ahead of the last and coolest catalyst bed can be problematic. Needs for complete consumption of oxygen are: 1) a burner that creates a highly turbulent, well-mixed flame; and 2) at least 0.5 seconds of residence time for the natural gas combustion products to react prior to mixing with the catalyst bed feed gas.
Note the increased residence time needed for combustion products from a natural gas-fired reheater (0.5 seconds) versus from an acid gas-fired reheater (0.2 seconds). Some plants with the capability to fire reheaters with either acid gas or natural gas have seen a rapid decrease in catalyst bed activity when switching from acid gas to natural gas. This is usually because the combustion chamber gives enough time for complete oxygen consumption when firing acid gas, but not when firing natural gas.
Soot can form if too little air is fed to a natural gas burner, causing off-color sulfur or plugging and deactivating the catalyst bed. The natural gas can be fed with steam (at 1-1.25 pounds of steam per 1 pound of gas) to help prevent soot formation.
1.3. Hot Gas Bypass Reheat
A hot gas bypass reheat usually requires a two-pass waste heat boiler. Some partially cooled gas out of the first boiler pass is bypassed to a reactor feed that needs heating. Bypassing directly from the thermal reactor is almost never completed because it is usually too hot to be completed reliably.
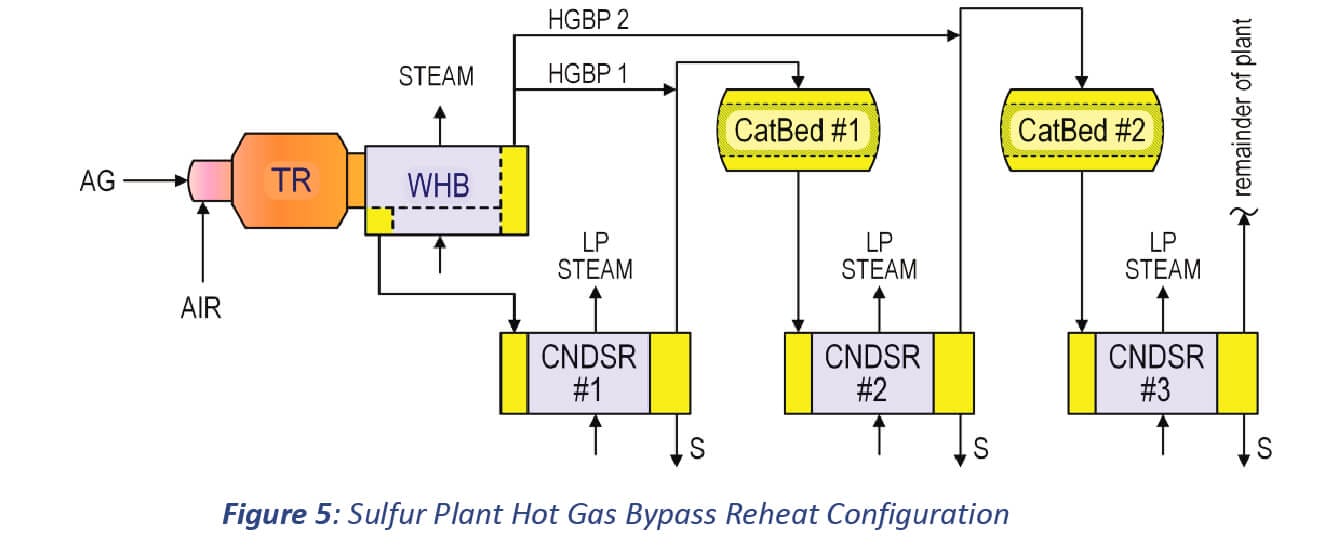
2. Indirect Reheaters
Indirect reheaters are more common in newer plants due to flexibility and reliability. They use steam, hot oil, or an electric current as a heat source or heat exchange with a hotter gas.
2.1. Steam Reheaters
Steam heats the feed gas to the catalyst beds.
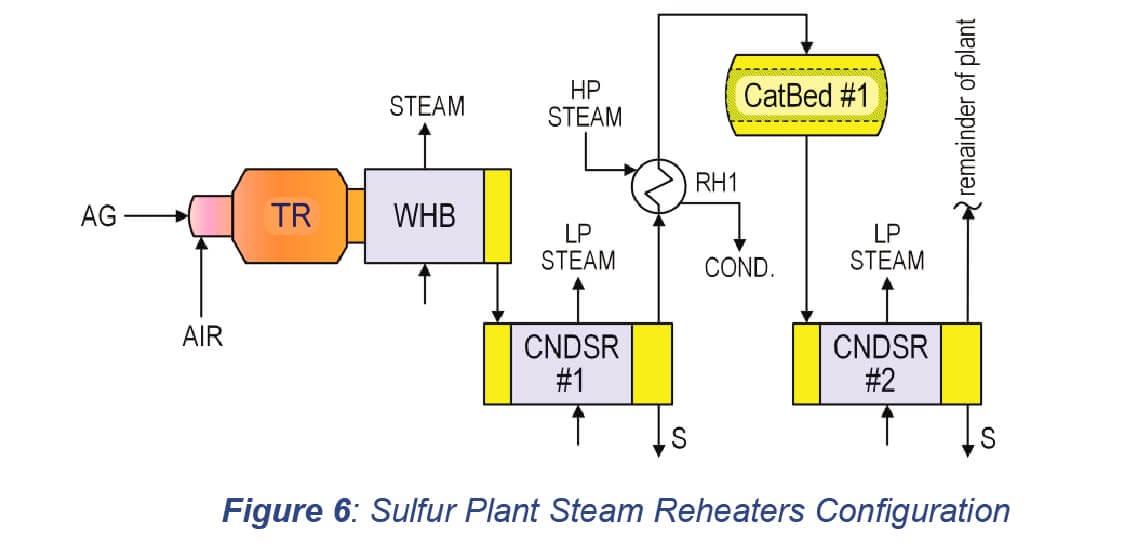
2.2. Electric Reheaters
Electric resistance reheaters are common in smaller plants. Electric heaters must be capable of supplying enough extra heat to compensate for losses. Thus, electric reheaters are commonly rated for 25% to 50% over the maximum process need.
2.3. Hot Oil Reheaters
Hot oil reheaters are often used in smaller plants or when available steam is of too low a pressure to supply the temperature needed. Hot oil reheaters must also be capable of compensating for losses, both in the hot oil system and the Claus process. The hot oil is usually a commercial heat transfer fluid but is sometimes hydrotreated diesel fuel.
2.4. Gas-Gas Heat Exchangers
Gas-gas heat exchangers generally use the hot effluent from the first reactor bed to heat the feed to downstream beds.
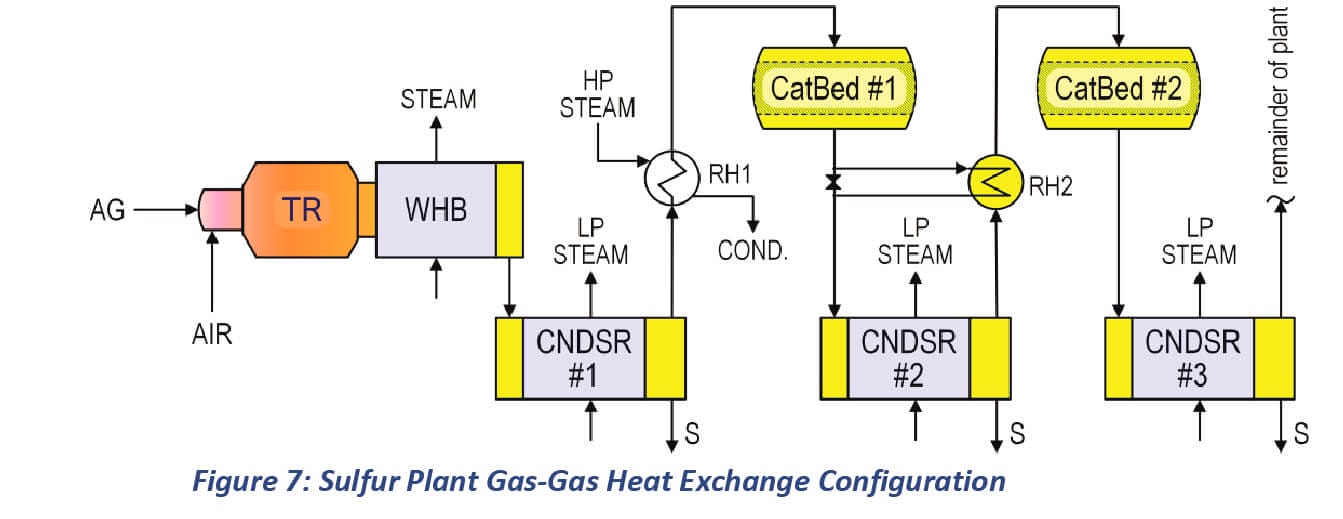
Ammonia Destruction
In addition to H2S-rich amine unit acid Gas, most refinery sulfur recovery plants can also take sour water stripper gas as a second feed. Sour water stripper gas typically contains roughly equal percentages of NH3 and H2S, a very low percentage of CO2 and is saturated with water vapor (30 to 40% H2O). Sour water stripper gas can also contain traces of hydrocarbons, phenol and HCN. Traces of ammonia can also be found in amine unit acid gas when there is insufficient water washing upstream of certain refinery amine contractors.
Ammonia can be destroyed in the thermal reactor. Ammonia reacts faster with SO2 than with O2, and H2S will react faster with the available O2 than ammonia. Hence, the dominant reaction for ammonia destruction is: 2 NH3 + SO2 → N2 + H2S + 2 H2O
Some ammonia destruction can also form elemental sulfur and hydrogen.
4 NH3 + 3 SO2 → 2 N2 + 6 H2O + 3/2 S2
4 NH3 + SO2 → 2 N2 + H2O + H2S + 4 H2
If excess molecular oxygen remains after reacting with H2S, some ammonia can react with it, forming NOX and leading to some SO3 formation.
2 NH3 + 2.5 O2 → 2 NO + 3 H2O
2 NO + O2 → 2 NO2
NO2 +SO2 →NO+SO3
No detectable NOX surviving out of the thermal reactor has been reported. This is likely because the NOX reacts with H2S and S2 in a downstream zone of the thermal reactor, where all O2 has been consumed.
There is little evidence for SO3 surviving out of the thermal reactor, because most SO3 reacts with H2S to form SO2 and S2, before exiting the thermal reactor.
At temperatures below about 350°F, surviving SO3 can combine with water vapor to condense into corrosive dilute sulfuric acid.
SO3 +(X)H2O→2H– +SO4–2 +(X-1)H2O
There are rare reports of acidic corrosion of the steel shell behind the refractory in the front portion of a thermal reactor, where the shell has been too cool. It may be possible that those thermal reactor’s acidic corrosion events occurred during “hot standby” operations, or during the later stages of shutdowns. During such periods, natural gas may have been burned with some excess air, and the remaining O2 may have oxidized traces of sulfur remaining in the refractory to SO2 and some SO3.
Residual ammonia from the thermal reactor at concentrations less than 150 ppmv is usually low enough to prevent downstream plugging due to the formation of ammonium salts. Such ammonium salt plugging depositions are usually sulfate and thiosulfate. Since SO3 and S2O2 are not known to make it out of the thermal reactor, their most likely source is from the catalytic reactors. H2SXOY acids are formed as intermediates in the catalyzed Claus reaction. These can lead to plugging deposits of ammonium sulfate and thiosulfate in the cooler sulfur condenser outlets of the second and third catalytic stages.
Two common thermal reactor configurations for destroying ammonia are a one-chamber thermal reactor and a two-chamber thermal reactor.
One-Chamber Thermal Reactor
Advantages of a One-Chamber Thermal Reactor
- All acid gas and sour water stripper gas contaminants (hydrocarbon, NH3, HCN and amine) pass through the flame, decreasing the possibility that they will escape the thermal reactor.
- The combustion is reducing (all O2 consumed), preventing NOX and SO3 formation.
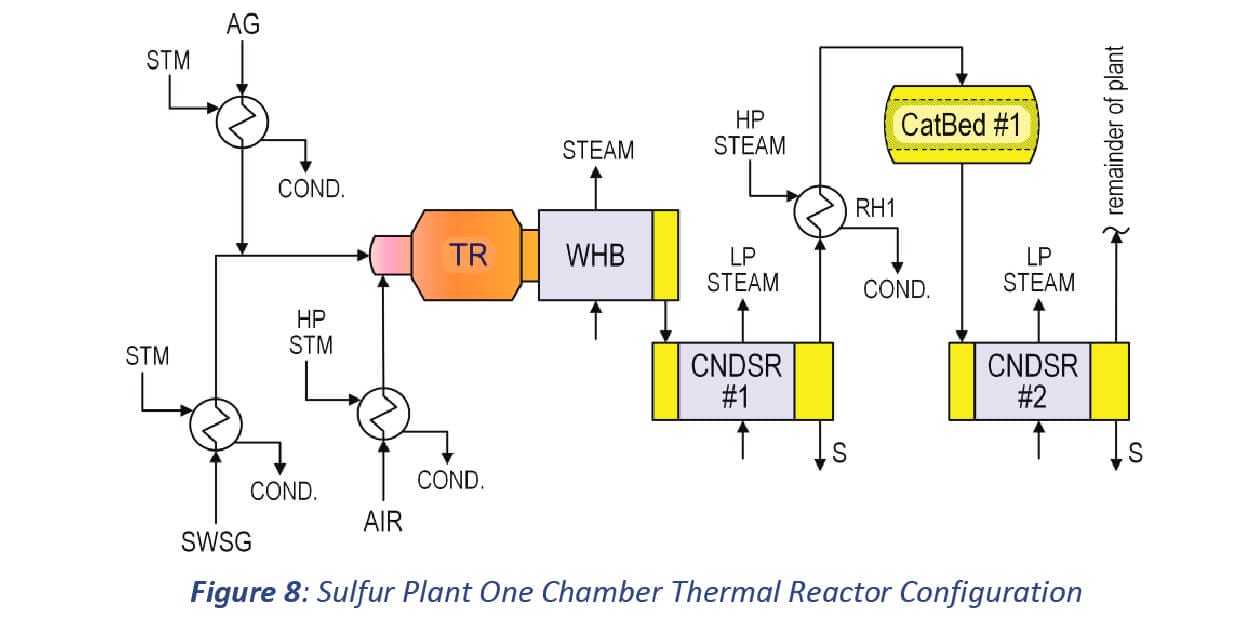
Disadvantages of a One-Chamber Thermal Reactor
- Flame temperature is determined by the compositions and temperatures of the acid gas, sour water stripper gas and combustion air feeds. To get sufficient temperature for NH3 destruction (at least 1260°C or 2300°F – actual, not adiabatic), considerable preheat of the acid gas, sour water stripper gas and air or oxygen enrichment of the air is needed.
- In some cases, it may be possible to add a little natural gas to the burner, in order to sufficiently raise the thermal reactor temperature. However, burning natural gas uses up some of the capacity of the sulfur plant, and will slightly reduce the percentage of sulfur recovered. In one investigation, adding enough natural gas to increase the thermal reactor temperature by 100°F increased the required combustion air by 18%, and reduced the sulfur recovery percentage by 0.4%.
Two-Chamber Thermal Reactor
Advantages of a Two-Chamber Thermal Reactor
- The front chamber temperature can be controlled directly by varying the fraction of acid gas bypassing the burner.
- The flame can be controlled to be either oxidizing or reducing.
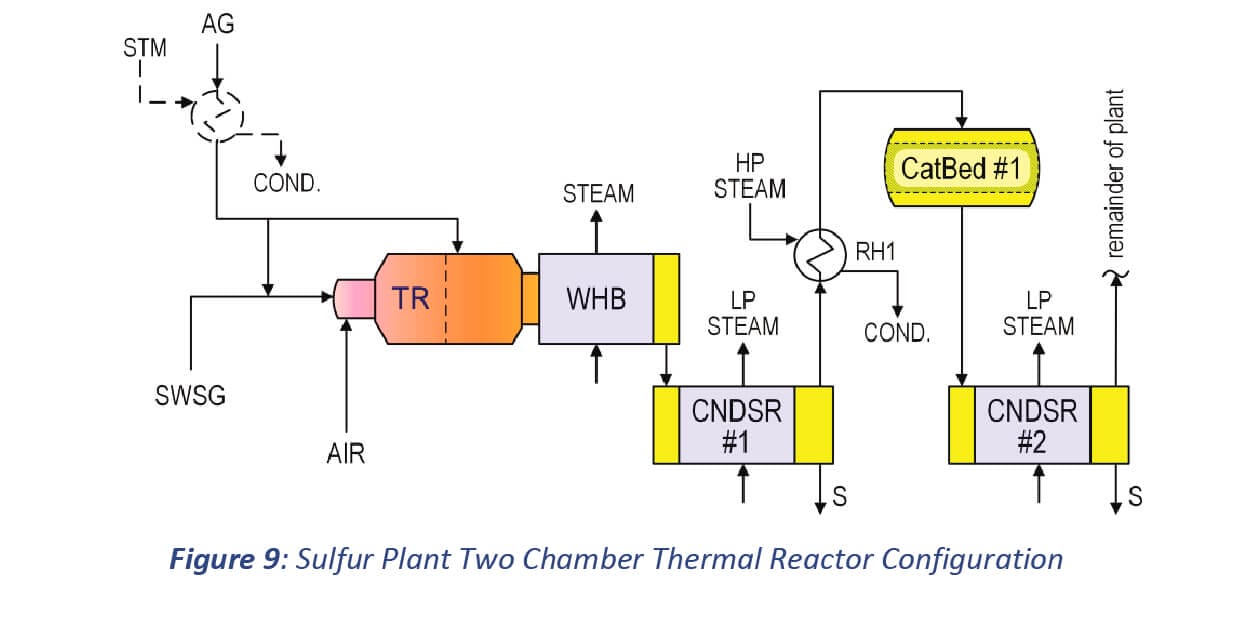
Disadvantages of a Two-Chamber Thermal Reactor
- Acid gas bypassing the flame to the second chamber does not go through an active flame and is not subjected to higher turbulence and temperatures. This could result in incomplete destruction of heavier hydrocarbons or aromatics in the acid gas as well as soot formation. Any traces of ammonia in the acid gas could also bypass the flame.
- Bypassed acid gas can have a relatively short residence time, which limits side reactions and can result in slightly higher COS and CS2 residuals.
- If the burner and first chamber are run in oxidizing mode (some O2 remains in the first chamber because more acid gas bypasses the second chamber), there is a possibility of some NOX and SO3 formation in the first chamber. As indicated above, such SO3 may result in corrosion issues.
Ammonia Destruction Parameters
Temperature – A thermal reactor requires 1260°C (2300°F) minimum actual or measured (not calculated adiabatic) for ammonia destruction.
Time – A thermal reactor requires a minimum of 0.8 seconds for a single chamber or 0.7 seconds for the first of a two-chamber thermal reactor. The second chamber should have a minimum of 0.4 seconds of residence time.
Turbulence – Highly turbulent burners that quickly mix combustion air and acid gas are desirable. This turbulence results in better use of the full thermal reactor volume, giving the maximum time for reactions to complete. A low-turbulence burner with a long flame front results in slower mixing and the bulk flow missing peripheral volumes outside the edges of the flame front. A refractory brick checker wall, or a Blasch VectorWallTM, separating the two thermal reactor chambers helps maintain somewhat higher flame temperatures by partially blocking radiant heat transfer from the flame to the boiler tube inlets. Such a refractory wall can also improve mixing in the second chamber.
Claus Unit Combustion Air Controls
Combustion air flow rate to the burner at the front end of the Claus unit is tightly controlled in order to make the optimum amount of SO2. About a third of the H2S is burned to SO2 and water. Then, the remaining H2S reacts with that SO2 to make elemental sulfur and water:
H2S+3/2 O2 →SO2 +H2O
2 H2S + SO2 ↔ 3/X SX + H2O, where x varies from 2 to 8 depending on temperature.
If the H2S and SO2 are balanced in a 2 to 1 ratio, the conversion to elemental sulfur, SX, is maximized.
The controlled value for the combustion air flow rate is determined by applying a multiplier, or ratio, to the acid gas feed flow rate. If there is also a sour water stripper gas feed, its flow rate is also multiplied by an appropriate ratio, and the result is added to the air needed for acid gas. The air-to-feed multipliers (or ratios) can be adjusted to achieve proper burning for those feeds.
For fine-tuning, an analyzer measuring mol% H2S and mol% SO2 is usually placed on the outlet gas piping from the final sulfur condenser. A control signal developed from that analyzer output is used to make a small adjustment to the combustion air flow rate. That adjustment can be applied as a correction to the air ratios for the acid gas and sour water stripper gas feeds. Alternatively, a “trim air flow” is adjusted through a smaller, separate control valve around the main air control valve. For adjusting the air-to-feed ratios, the output of the analyzer controller is a multiplier ranging from about 0.92 to 1.09. For trim air control, the trim air flow rate should normally be about 10% of the total air flow rate at design capacity, with the capability to range from about 3.5% to 17%.
Although the goal of the analyzer controller is to achieve a 2 to 1 ratio of %H2S to %SO2, that ratio is not proportional to the air flow rate. A term referred to as excess H2S is closely proportional to (or linear with) air flow rate:
%excess H2S = % H2S – 2*(% SO2)
Thus, when (%H2S)/(% SO2) = 2.0, then %excess H2S = 0.0. Controlling air to achieve zero excess H2S gives a 2 to 1 ratio of H2S to SO2.
Some control systems are set up to give zero “%air demand.” %air demand is the percentage that air needs to be increased to give a 2 to 1 ratio of H2S to SO2. %air demand is proportional to %excess H2S. Usually, about 3.0 * %excess H2S = %air demand.
Claus unit air controls are not always set to target a final 2 to 1 ratio of %H2S to %SO2. When a tail gas unit downstream of the Claus unit includes a tail gas hydrogenation reactor, the ratio of %H2S to %SO2 is often set between 4 and 8. Depending on sulfur plant feed composition and recovery, this 4 to 1 through 8 to 1 ratio can be achieved by controlling an excess H2S of 0.2% to 0.3%.
A higher H2S to SO2 ratio may slightly decrease Claus unit sulfur recovery, but it will result in a smaller concentration of SO2 going into the tail gas unit. Hydrogenation of the SO2 in the tail gas reactor consumes most of the hydrogen and is the largest contributor to increased temperature in that reactor. Minimizing SO2 out of the Claus unit minimizes the chance of too much SO2 going into the tail gas unit during upsets. Unreacted SO2 escaping the tail gas reactor can result in plugging and corrosion in downstream equipment and can result in the degradation of the tail gas unit amine.
Tail Gas Units
Tail gas exiting a Claus sulfur plant is usually sent through further processing to minimize emissions of sulfur compounds. This processing can be done either before or after incineration of the tail gas. There have been dozens of tail gas treatment processes postulated over the last 50 years. Two broad classes of processes in current use are briefly discussed below.
1. Incinerate and Scrub
The sulfur plant’s tail gas is incinerated to oxidize sulfur compounds to SO2, then contacted with a caustic solution process to remove the SO2 prior to venting. The spent caustic is a waste that must be responsibly disposed of.
DynaWave® (now licensed by Elessent) scrubbing technology is highly efficient for caustic scrubbing of incinerated sulfur plant tail gas. Usually, features are incorporated to oxidize residual traces of reduced sulfur compounds in the spent caustic. There are many DynaWave® units around the world that handle sulfur plant tail gases and other gases.
Some DynaWave® installations scrub incinerated tail gas without any heat recovery prior to the scrubbing. Others incorporate a waste heat boiler ahead of caustic scrubbing to produce high-pressure steam and reduce cooling and circulating flow requirements for the scrubbing solution. Incineration at higher temperatures for the destruction of CO generally includes a waste heat boiler ahead of scrubbing.
In some refineries, the Fluid Catalytic Cracking unit (FCC) wet gas scrubbing unit (such as a Belco® Unit) has sufficient excess capacity to accept incinerated SRU tail gas. The tail gas incinerator is generally followed by a waste heat boiler prior to routing to the wet gas scrubber.
Up to 3% of the SO2 formed when incinerating sulfur compounds can oxidize further to SO3. This SO3, along with the water vapor present in any SRU tail gas, can result in an acidic dew point as high as 350°F. Diluted sulfuric acid could begin condensing on surfaces cooler than that dew point. Steam at 120 psig condenses at 350°F. For a 50°F margin above the acidic dew point, it is commonly recommended that the waste heat boiler produces steam no lower than 400°F (235 psig) to protect the boiler tubes and outlet piping from sulfuric acid corrosion.
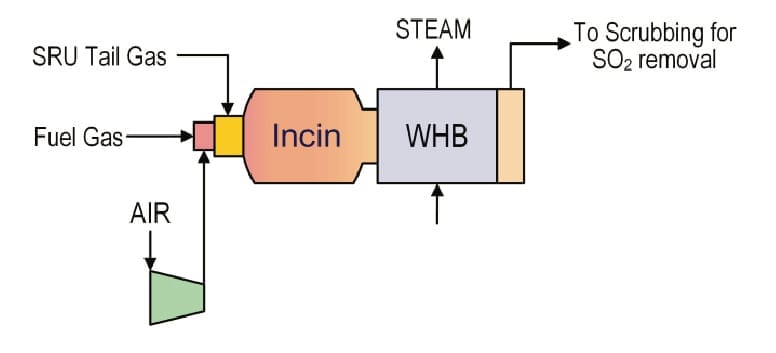
The FCC wet gas scrubber in a refinery is often a significant distance from the sulfur recovery facilities. It is essential that the piping between the incinerator waste heat boiler and the wet gas scrubber be kept hot. Tracing with steam of at least 235 psig may be required for the piping and pipe supports.
2. React, Absorb and Recycle
A commonly used method for cleaning up tail gas is to convert all sulfur compounds exiting with the Claus Unit tail gas to H2S (React). The reactor effluent is then cooled and contacted with a selective amine to absorb that H2S. That amine is then regenerated in a typical amine stripper tower, and the stripper overhead acid gas is recycled to the sulfur recovery unit.
This strategy was first used in units licensed by Shell Oil Co. (SCOT units). Many other facilities using this strategy have been designed and built with available non-licensed technology and methods.
2.1. Reacting Claus SRU Tail Gas
Claus SRU tail gas must be heated, have a reducing gas added and be routed through a catalyst bed where the various sulfur compounds are converted to H2S. Reactions in the catalyst bed are summarized as follows:
SO2 hydrogenation to H2S and water vapor. This reaction essentially goes to completion in the normal operating temperature range for a catalyst that is reasonably active: SO2 + 3 H2 → H2S + 2 H2O
Elemental sulfur hydrogenation. “n” here is usually between 2 and 6 at the catalyst temperature. This reaction essentially goes to completion at a normal operating temperature range for a catalyst that is reasonably active: Sn + n H2 → n H2S
COS hydrolysis. Equilibrium favors COS conversion at lower temperatures: COS + H2O ↔ CO2 + H2S
CS2 hydrolysis. Equilibrium favors CS2 conversion at lower temperatures: CS2 + 2 H2O ↔ CO2 + 2 H2S
Water gas shift equilibria. Due to this reaction and an abundance of water vapor in SRU tail gas, CO also serves as a reducing gas by making H2: CO + H2O ↔ CO2 + H2
To ensure that sufficient hydrogen and CO are available, historic or predicted Claus SRU recovery is commonly reduced by 2 to 3% for design purposes. This conservative deduction from sulfur recovery generally depends on experience with the SRU or with the facility in which the SRU will be installed. For example, an SRU in a complex refinery with a sour water stripper should probably have a larger recovery deduction than an SRU in a gas processing facility. Some designers deduct 2% from the guaranteed SRU-only recovery. This assumed recovery deduction should be implemented via the reverse Claus reaction:
3/n Sn + H2O → 2 H2S + SO2 (elemental sulfur, Sn, here is deducted from recovery)
The design rate of reducing gas to be added should be enough so that the predicted effluent from the catalyst bed (with the assumed deduction in sulfur plant recovery) contains no less than 1.5 to 2% H2 + CO on a wet basis.
The SRU tail gas is first heated before reaching the catalyst bed. This is commonly done by direct firing, indirectly heating with hot oil or indirectly heating with electric resistance.
The tail gas unit catalyst is usually in a separate reactor vessel that is fully refractory-lined. Common feed temperatures for this bed range from 485°F to 525°F. The catalyst is similar to a naphtha hydrotreating catalyst and is usually a sulfided cobalt-molybdenum catalyst on activated alumina support balls or pellets.
Some newer catalysts can allow feed temperatures as low as 430°F to 450°F, which could allow the use of 600 psig steam (489°F at saturation) for indirect feed heating. However, increasing catalyst feed temperature can restore the activity of an aging catalyst. Also, if a non-sulfided catalyst is loaded into the reactor (the cobalt and molybdenum are oxides), an in situ sulfiding procedure typically requires heating the entire catalyst bed to at least 600°F.
A. Direct Fired Feed Heating
Any propane and lighter hydrocarbon gas of constant composition can be suitable as a fuel used for direct heating of SRU tail gas. The preferred fuel is natural gas. The fuel gas is burned with less than 90% of stoichiometric air. A flame starved for air is needed to prevent molecular oxygen from escaping the flame. Such oxygen can slowly sulfide the activated alumina support of the downstream catalyst, causing that catalyst to lose activity. Because less oxygen is supplied than is needed to convert the gas to CO2 and water, some CO and H2 are made. Rarely is air used at less than about 80% of stoichiometric, but some installations run as low as 70% in order to supply more CO and H2 as reducing gas. Too little air will result in soot formation, which can increase catalyst bed pressure drop, decrease catalyst bed activity and possibly plug downstream quench water filters. Steam can be fed with natural gas (at 1 to 1.25 lb steam per 1 lb natural gas) to help prevent soot formation. Generally, a high-quality burner with air spin vanes is used, which promotes quick turbulent mixing of the burning gas and the air.
Usually, the combustion chamber is designed for about 0.5 seconds of burner combustion product residence time. At the end of the combustion zone is a choke ring or checker wall. Beyond that, the combustion products mix with the SRU tail gas and any hydrogen-reducing gas.

Hydrogen may need to be added downstream of the combustion flame if the flame does not generate enough CO and H2. It is recommended that sufficient reducing gas be present so that the effluent from the catalyst bed contains no less than 1.5 to 2% H2 + CO.
When a direct fired heater is relied upon to make all supplemental reducing gas (no external H2 is added), the SRU tail gas can often be heated to a higher temperature than desired for the TGU CatBed feed. In that case, the effluent from the heater needs to be cooled. Most often, such cooling is done in another tube pass in the shell of the waste heat boiler. A partial bypass around this tube pass can be adjusted to achieve the desired reactor feed temperature. Sometimes, the reactor feed cooling tubes are placed in a separate shell in order to generate high-pressure steam.
B. Indirect Reactor Feed Heating
Indirect reheating is normally done with hot oil or electric resistance. The direct fired reactor feed heating diagram in Figure 11 above simplifies down to the Figure 12 diagram below when using indirect heating. Note that hydrogen from an external source is used to reduce gas.
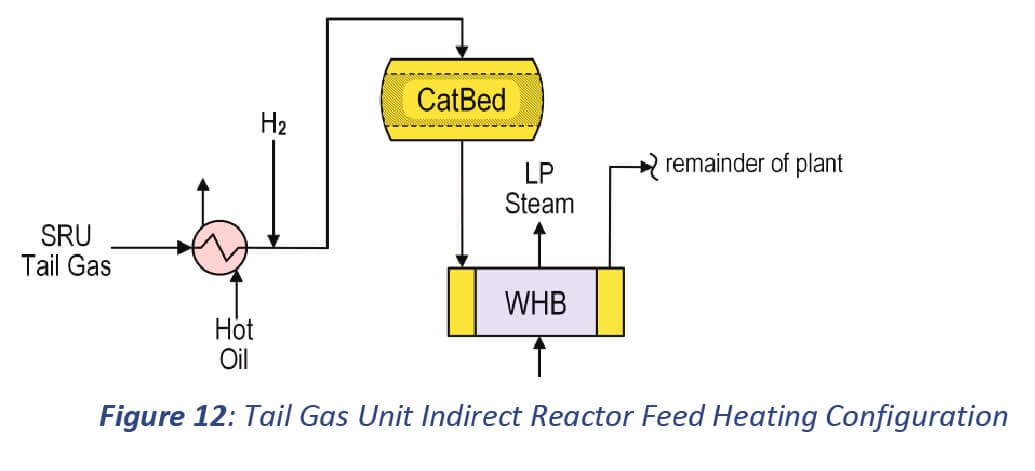
A note on external reducing gas: Hydrogen from a naphtha reformer can successfully serve as reducing gas to a TGU reactor. However, the hydrogen must be sufficiently cooled in the reforming unit to condense heavier hydrocarbons, and any liquids should be removed ahead of the TGU. Otherwise, benzene, other aromatics, unsaturated hydrocarbons and C5+ hydrocarbons can be fed to the TGU reactor, which will deactivate the catalyst. Hydrogen from a unit based on steam-methane reforming should not have such problems.
2.2. Cooling of Reacted Gas
A. Waste Heat Boiler
Hot gas from the reactor first proceeds through a waste heat boiler. Hot gas usually proceeds through the tubes with boiling water in the shell. The waste heat boiler is usually built like a sulfur condenser, but the outlet has no provisions for liquid sulfur separation and drainage. The waste heat boiler usually produces 40-60 psig steam. The target outlet gas temperature is 315°F to 325°F. Lower outlet temperatures result in less quench water cooling downstream.
Some larger plants have two stages of waste heat boiling downstream of the catalyst bed: first cooling the gas by making 200 to 300 psig steam, followed by further cooling to produce 40-60 psig steam.
Some dual waste heat boilers are configured with the hot gas in a plenum, flowing horizontally past two sets of vertical finned tubes. Each tube set connects to its own steam drum above and mud drum below. This arrangement can result in a lower pressure drop for the hot gas but will be more expensive.
In some plants, a gas-gas heat exchanger uses hot reactor effluent to help heat the reactor feed.
B. Quench Loop
Gas out of the waste heat boiler (315 – 325°F) is too hot for contact in an amine absorber, so further cooling is needed. This gas should be scrubbed with water to absorb any last traces of SO2, which will degrade the amine. The quench loop consists of a quench tower, quench water circulation pumps, a quench cooler and filtration.
Gas from the waste heat boiler enters the bottom of the quench tower. It then proceeds upwards and is contacted and cooled by quench water proceeding downwards. The contacting occurs in a 9 to 15-foot-tall randomly packed bed of slotted stainless steel rings, or through about six stationary valve trays. This cooling condenses most of the water in the gas.
Warm water from the bottom of the quench tower is pumped to either air coolers, water coolers or air followed by water. Cooled quench tower bottom water is then fed back to the top of the quench tower.
A side stream of 20 to 25% of the pumped water is commonly diverted through particulate filters. Since water is condensed in the quench tower, some of the filtered water is rejected to sour water collection to maintain a steady level at the bottom of the quench tower. The remainder of the filtered water returns to the pump suction.

An analyzer monitors the pH of the circulating quench water, usually downstream of the cooler. Any breakthrough of SO2 from the catalyst bed will decrease the quench water pH.
- The quench water pH should normally be about 6.5 to 7.5.
- pH values lower than about 6.0 can result in traces of SO2 escaping the quench tower and entering the downstream amine absorber. SO2 degrades the amine, decreasing its H2S absorption capacity and making it more corrosive.
- Severe SO2 breakthrough from the reactor can react with H2S also present, to form finely divided solid elemental sulfur in the quench water. Such sulfur can lead to very rapid plugging of the filters, plugging of pump suction screens, and plugging of any mesh pad mist eliminator in the top of the quench tower.
An increase in pH is best remedied by looking upstream of the quench tower.
- Too much combustion air to an upstream Claus SRU will result in an H2S to SO2 ratio of less than 2 to 1. If this ratio is low, it means more SO2 than needed for optimal recovery is escaping to the tail gas unit. SRU air controls need to be adjusted so that the H2S to SO2 ratio is 2.0 or greater (%excess H2S is 0.0% or greater).
- Burning with excess air in a direct-fired feed heater (either in the tail gas unit or in the SRU) can result in oxygen escaping the flame. This oxygen will combust with hydrogen and CO in the tail gas unit catalyst bed, increasing catalyst bed temperature and possibly allowing unreacted SO2 to escape to the quench tower.
Fixing upstream issues that decrease pH may take time and may leave a large inventory of low-pH quench water in equipment and piping. Therefore, a means is usually provided for injecting a caustic solution to neutralize the circulating quench water. Alternatively, if an operator prefers not to deal with caustic, pH can be adjusted by quickly dumping the quench water and replacing it with a source of clean water.
2.3. Absorbing H2S with a Selective Amine
A. Absorber
Cooled gas from the quench tower overhead is routed to the bottom of a trayed or packed absorber tower. A selective amine solution, most commonly a 30 to 50 wt% methyl-diethanol amine (MDEA) solution, enters this absorber above the trays or packing and absorbs most of the H2S while allowing most of the CO2 to escape to the absorber overhead gas. Other selective amines used are di-isopropanol amine (DIPA) and various proprietary, licensed formulations.
This absorber generally contains 10 to 15 valve trays. Tray spacing is often 30″ rather than a more typical 24″. The larger tray spacing usually allows for a decrease in tower diameter, thereby decreasing the residence time of the amine on the trays. This lower residence time tends to decrease the amount of CO2 absorbed due to the slower rate of CO2 absorption. Instead of trays, some absorbers have 25 to 30 ft. of random (slotted ring) stainless steel packing, generally in two beds.
Trayed absorbers are generally less susceptible to foaming issues and will allow a greater reduction of amine flow rate at reduced tail gas throughput. However, many packed absorbers have performed quite well, and packed absorbers can also be somewhat more selective toward H2S absorption over CO2 absorption. For packed absorbers in tail gas service, it is particularly important to have good liquid distribution above the beds and good vapor distribution below the bottom bed.
B. Filtration
The extent and configuration of filtration for the selective amine varies.
The following diagram with the selective amine absorber shows full-stream particulate filtration of the lean amine, followed by approximately 20% of that filtered amine going through an activated carbon adsorber/filter. Some may question the justification for an activated carbon bed since there is no hydrocarbon content in the feed to a tail gas absorber. However, activated carbon can absorb some amine degradation products, capture some of the smaller iron sulfide particles passing through the main filter, and generally decrease the foaming tendency.
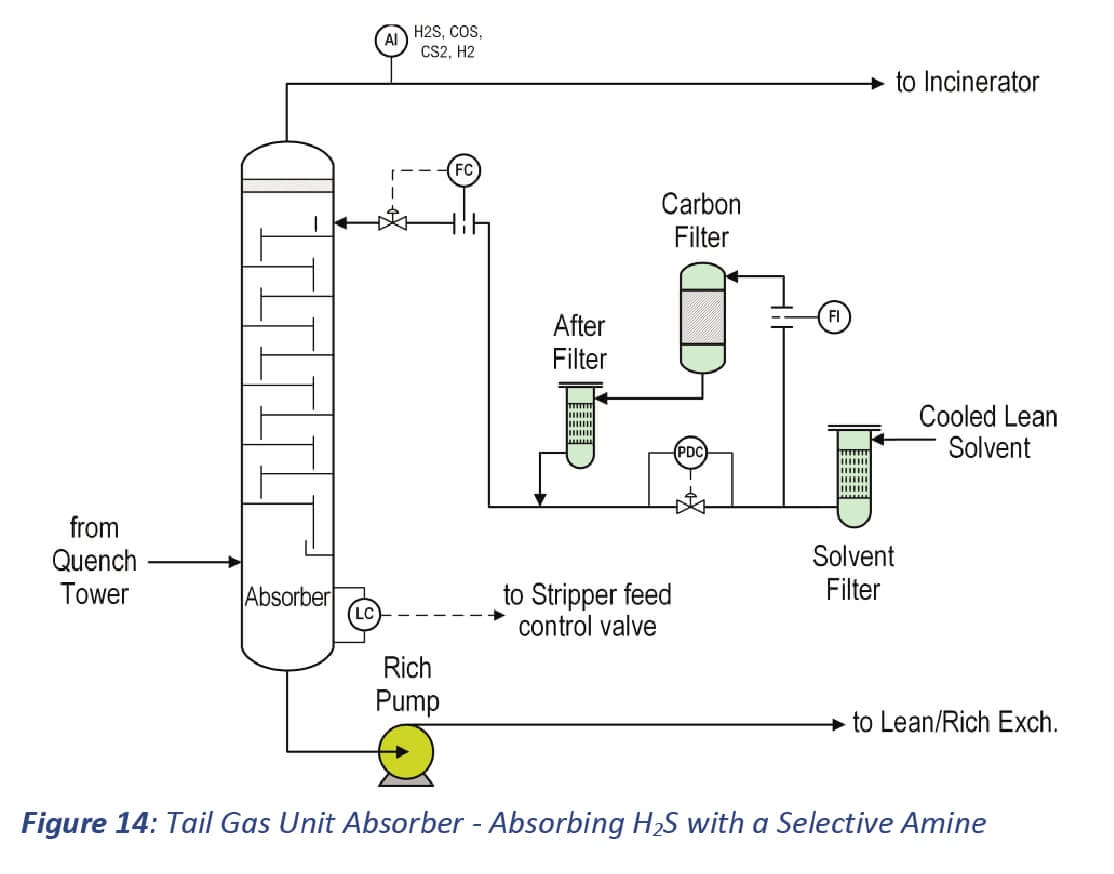
Many tail gas units have less extensive amine filtration schemes than shown in the above diagram. Some units have no carbon filter. Occasionally, only 20% of the amine stream is sent through particulate filtration.
Some operators prefer to place particulate filtration on the H2S-loaded rich amine from the absorber. This is because some iron sulfide can dissociate in the stripper tower to form soluble iron compounds. Tail gas unit-rich amine is not highly loaded with H2S (rarely exceeding 0.10 mol H2S / mol amine) and forms less iron sulfide in steel equipment and piping exposed to that rich amine. For this reason, lean amine filtration usually works well in tail gas units. Changing and maintaining lean amine filters is also less hazardous than for rich amine filters.
2.4. Regenerating the Rich Amine, and Recycle of Acid Gas to the SRU
The amine regeneration system for a tail gas unit is very similar to most amine regeneration systems, except that there is no need for a rich amine flash drum. The main components are the lean/rich exchanger, stripper tower, overhead condenser, reflux drum, reboiler, lean amine cooler, reflux pump and lean amine pump. Auxiliary equipment can include an amine drain drum and pump and a lean amine storage tank and pump.
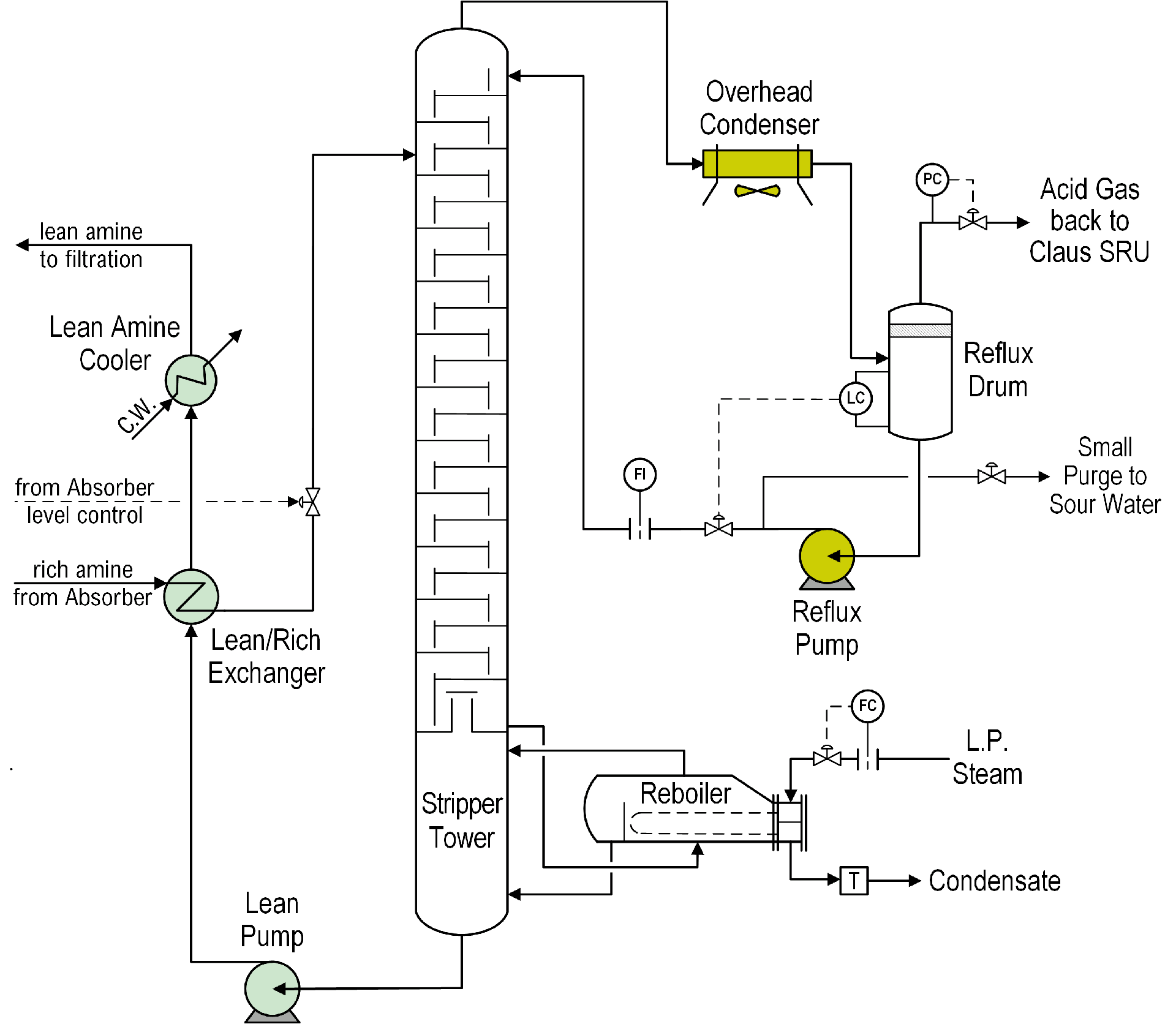
A. Lean/Rich Exchanger
The lean/rich exchanger cools the lean amine while heating the rich amine feeding the stripper tower. The amine should not be heated more than the temperature at which H2S starts vaporizing out of the rich amine at the tower feed pressure. This can usually be accomplished in two AEU or BEU shells in series. The rich amine is usually on the tube side, and the lean amine is on the shell side. Some tail gas units have hairpin or plate & frame lean/rich exchangers.
B. Stripper Tower
On average, the stripper tower has about 24 valve trays below the rich amine feed and three valve trays above the rich amine feed. The number of trays below the feed can range from 20 to 30, and the number of trays above the feed can range from two to four. Common tray spacing is 24”. A chimney tray or trap-out pan below the bottom tray collects feed to the reboiler. The average height of a stripper tower is about 70 feet from seam to seam. Rich amine traveling down the trays is heated by hot gas (mostly steam) rising from the reboiler. Acid gas and steam rising above the feed are washed by sour reflux water proceeding down the trays, which reduces amine loss to the tower overhead.
C. Overhead Condenser, Reflux Drum and Reflux Pump
Hot vapor (acid gas and steam) from the top of the stripper tower goes to the overhead condenser, which can be cooled by either air or water. Steam condenses to sour water during cooling in the overhead condenser. A two-phase stream exits the condenser and enters the reflux drum, where it is separated. The acid gas goes overhead to be recycled to the sulfur plant. The sour water out of the reflux drum is pumped back to the stripper as reflux. Usually, about 1% to 2% of the reflux is purged to limit the buildup of impurities and lighter amine degradation products.
D. Reboiler
Hot amine from the stripper tower bottom tray goes to the reboiler. Generally, 45 to 60 psig steam supplies heat to the reboiler at a rate of 850 to 1000 btu per gallon of amine circulated. The above flow diagram indicates a kettle reboiler (AKU or BKU), where vapor from the reboiler shell (mostly steam) enters the stripper below the chimney tray and liquid from the reboiler shell enters the tower bottom below the liquid level. Other reboiler types used in tail gas units are horizontal thermosyphons with steam in the tubes and vertical thermosyphons with steam in the shell. Thermosyphon reboilers in amine regeneration service should be fed all the liquid from the bottom tray, and they return a two-phase outlet stream to the stripper tower below the bottom tray. That is a “once-through” configuration, meaning no liquid out of the reboiler is added back into the reboiler feed. The sizing of the thermosyphon reboiler and piping must result in a pressure drop low enough to ensure that no liquid bypasses the reboiler. Such bypassing results when the amine backs up and overflows the bottom trap-out tray or the chimney tray’s chimney.
E. Lean Amine Pump
The above flow diagram shows the lean amine pump taking suction from the bottom of the stripper tower. The bottom of the stripper is a 255°F – 260°F bubble point liquid. Such pumps with adequate NPSH and metallurgy can serve well and have low maintenance. Some lean amine pumps take suction from the lean/rich exchanger shell outlet.
F. Lean Amine Cooler
The lean amine cooler can be air-cooled, water-cooled, or air-cooled followed by a water trim cooler. Keep in mind that a lower lean amine temperature will result in a lower residual H2S content of the absorber overhead. Also, a lower lean amine temperature usually results in better selectivity (less co-absorbed CO2). However, the lean amine should be no cooler than about 80°F. Higher liquid viscosity and other factors can slow H2S absorption at lower temperatures.